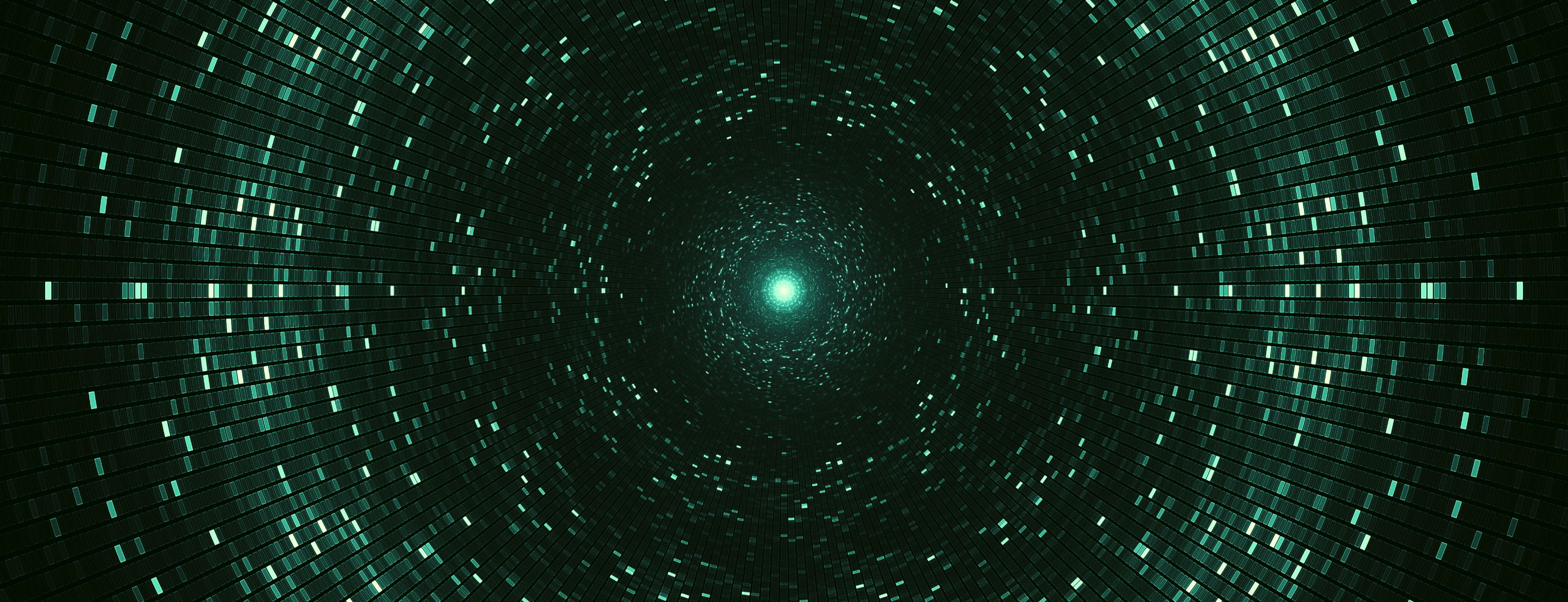
What is Nuclear Fusion?
The definition of nuclear fusion is the encounter of two light atomic nuclei that combine to form a heavier nucleus, releasing vast amounts of energy in the process. The main examples of nuclear fusion is the Sun and other stars that are powered by it. On Earth, scientists aim to replicate this natural process to harness clean and abundant energy.
Nuclear fusion differs from the nuclear fission used in current power plants. Fission involves splitting heavy atoms like uranium, while fusion merges light atoms, typically hydrogen isotopes—deuterium and tritium—to create helium, releasing energy in the form of heat and high-energy particles. This reaction is highly efficient, producing much more energy than fission for the same amount of fuel.
Nuclear fusion energy holds breakthrough potential.
Unlike fission, it generates no long-lived radioactive waste and carries no risk of a runaway reaction preventing any affect on air or biodiversity. With virtually limitless fuel supplies from seawater (deuterium) and lithium (tritium), nuclear fusion could provide a clean, safe, and sustainable energy source for humanity.
But achieving controlled fusion on Earth is a formidable challenge. Nuclear fusion requires extremely high temperatures and pressures—much like those at the core of stars. That’s where technologies like Inertial Confinement Fusion (ICF) come in.
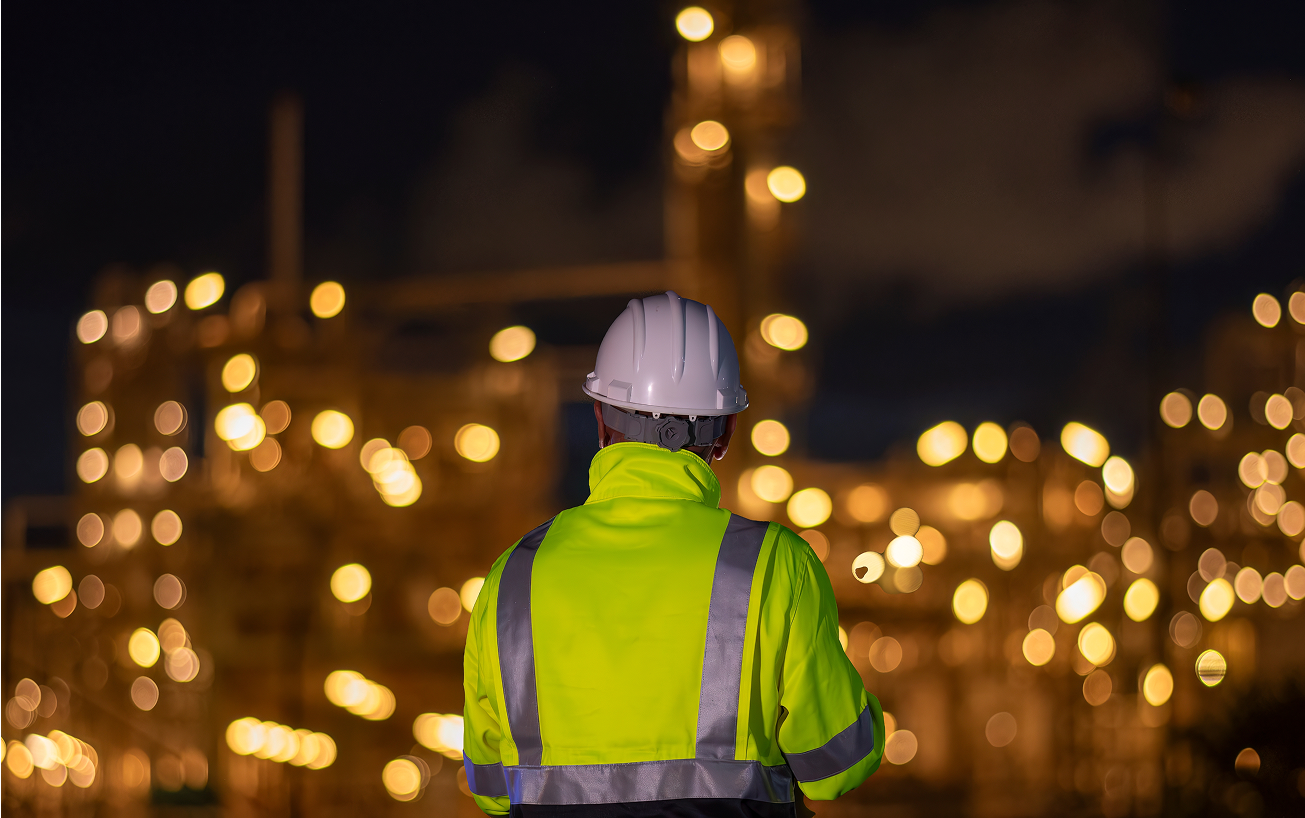
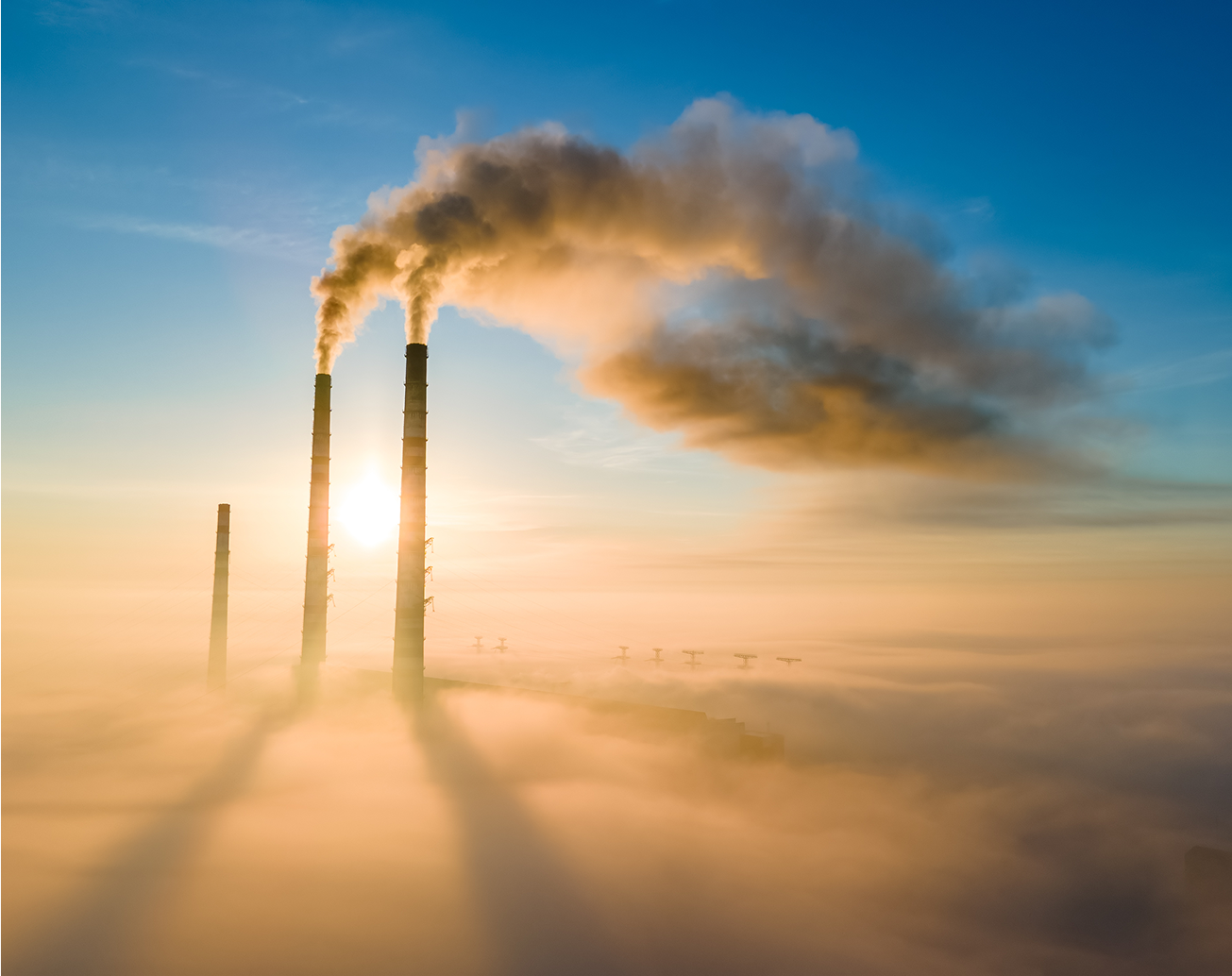
What is the definition of nuclear fusion?
At the atomic level, nuclei are composed of protons and neutrons. These particles are bound together by the strong nuclear force. However, in order for nuclear fusion to occur, the nuclei must overcome their natural electrostatic repulsion (because protons are all positively charged). To do this, the particles must be brought close enough for the strong nuclear force to take effect, but this requires extreme temperatures and pressures.
In stars like the Sun, this happens naturally due to the balance between gravity and nuclear fusion radiative pressure equations. The immense pressure and temperature at the core—around 15 million degrees Celsius—force hydrogen nuclei together, allowing them to fuse and produce helium and energy. For fusion to happen on Earth, we need to replicate these conditions artificially, achieving temperatures of around 100 million degrees Celsius and creating pressures millions of times greater than Earth’s atmosphere.
Achieving nuclear fusion with Lasers: Inertial Confinement Fusion (ICF)
One of the most promising approaches to achieving fusion on Earth is Inertial Confinement Fusion (ICF). This method involves using powerful laser beams to compress a tiny fuel pellet to the extreme conditions necessary for nuclear fusion.
Let’s break down the key components of ICF:
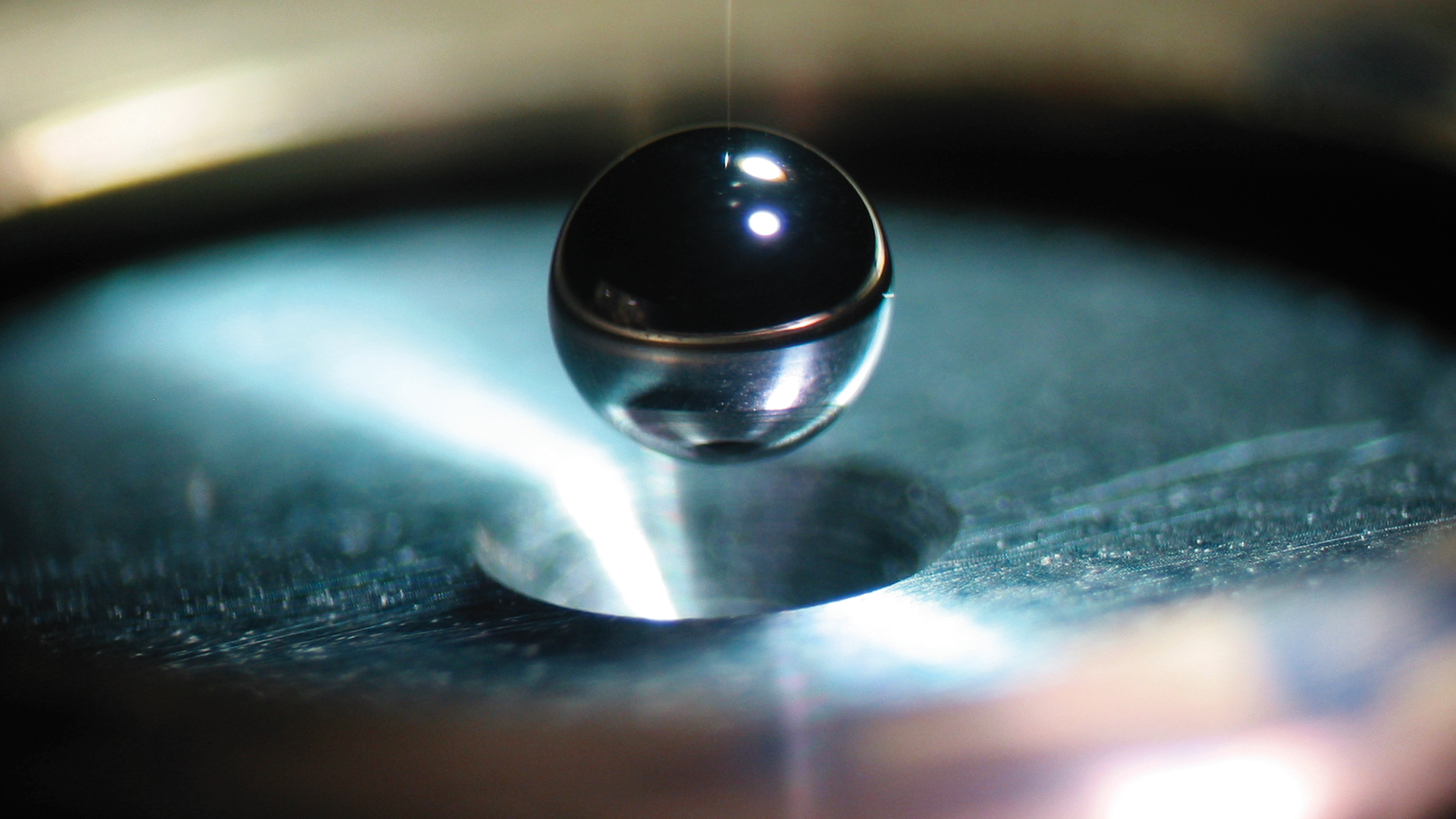
The Fuel Pellet
The fuel for ICF is typically a small, spherical pellet, just a few millimeters in diameter, made of deuterium and tritium, two isotopes of hydrogen. These are ideal for nuclear fusion because they have a lower Coulomb barrier, meaning less energy is required to overcome their natural repulsion.
The pellet is surrounded by a protective shell made of a material called an ablator (typically plastic or other lightweight materials). The purpose of the ablator is twofold: it absorbs the energy from the lasers and helps compress the fuel during the fusion process. When the lasers hit the ablator, it heats up rapidly, causing it to vaporize and create an explosive force that pushes the remaining fuel inward, compressing it to hundreds of times the density of solid lead.
(source : NIF / LLNL)
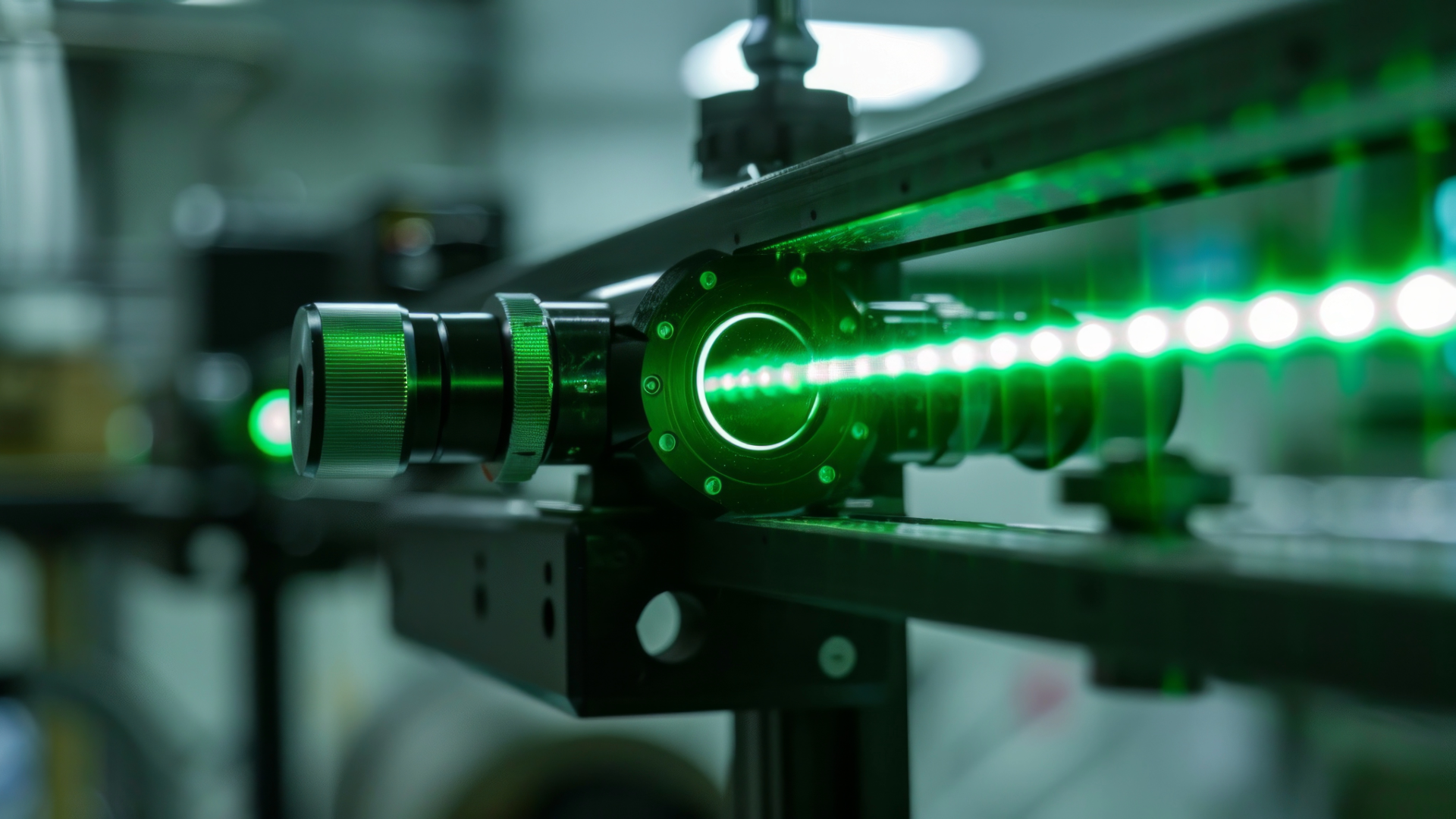
Laser Beams
The lasers used in ICF are powerful devices capable of emitting extremely intense energy in a very short period of time (often just a few billionths of a second). At research facilities like the National Ignition Facility (NIF) or the Laser Mégajoule (LMJ), hundreds of laser beams are directed at the fuel pellet, each carefully synchronized to ensure uniform compression.
The goal is to heat the outer layer of the pellet to millions of degrees, causing it to explode outward. This explosion generates an inward implosion, compressing the fuel and raising its temperature and pressure to the levels needed for nuclear fusion to occur. The energy from these lasers must be delivered precisely and symmetrically to ensure the fuel compresses evenly, as any asymmetry can lead to an inefficient fusion process.
Achieving nuclear fusion with Lasers: Inertial Confinement Fusion (ICF)
One of the most promising approaches to achieving fusion on Earth is Inertial Confinement Fusion (ICF). This method involves using powerful laser beams to compress a tiny fuel pellet to the extreme conditions necessary for nuclear fusion.
Let’s break down the key components of ICF:
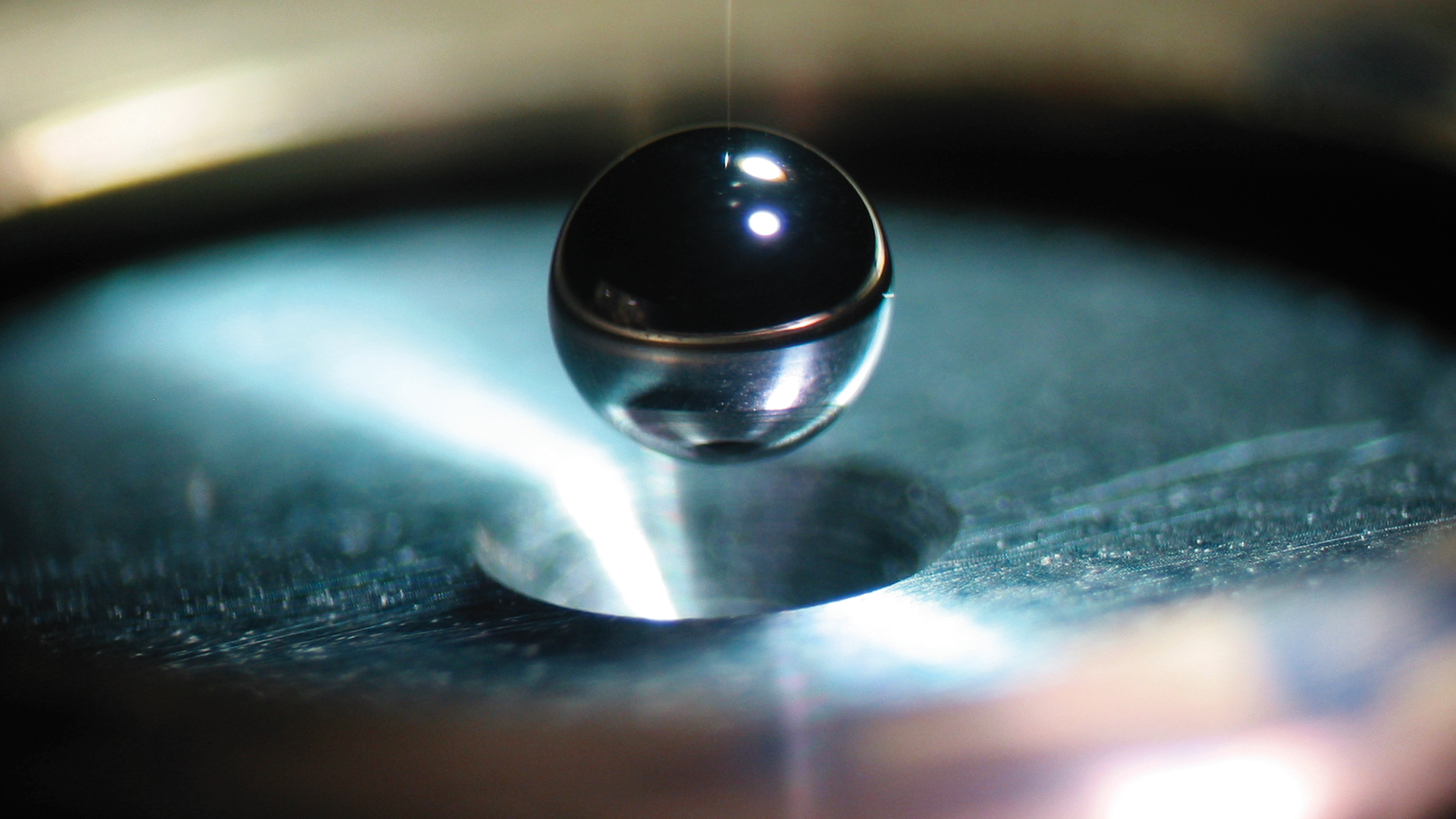
The Fuel Pellet
The fuel for ICF is typically a small, spherical pellet, just a few millimeters in diameter, made of deuterium and tritium, two isotopes of hydrogen. These are ideal for nuclear fusion because they have a lower Coulomb barrier, meaning less energy is required to overcome their natural repulsion.
The pellet is surrounded by a protective shell made of a material called an ablator (typically plastic or other lightweight materials). The purpose of the ablator is twofold: it absorbs the energy from the lasers and helps compress the fuel during the fusion process. When the lasers hit the ablator, it heats up rapidly, causing it to vaporize and create an explosive force that pushes the remaining fuel inward, compressing it to hundreds of times the density of solid lead.
(source : NIF / LLNL)
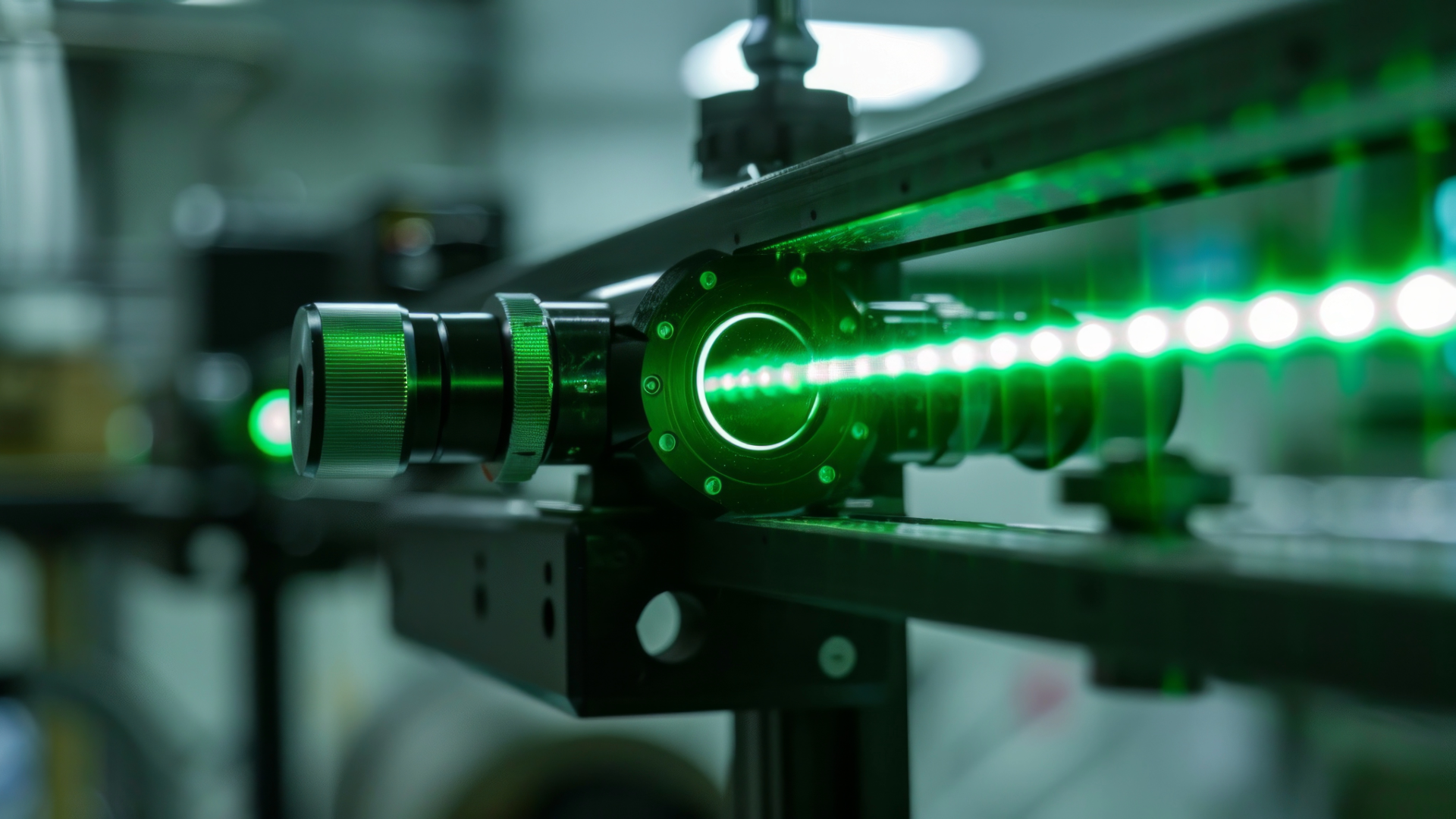
Laser Beams
The lasers used in ICF are powerful devices capable of emitting extremely intense energy in a very short period of time (often just a few billionths of a second). At research facilities like the National Ignition Facility (NIF) or the Laser Mégajoule (LMJ), hundreds of laser beams are directed at the fuel pellet, each carefully synchronized to ensure uniform compression.
The goal is to heat the outer layer of the pellet to millions of degrees, causing it to explode outward. This explosion generates an inward implosion, compressing the fuel and raising its temperature and pressure to the levels needed for nuclear fusion to occur. The energy from these lasers must be delivered precisely and symmetrically to ensure the fuel compresses evenly, as any asymmetry can lead to an inefficient fusion process.
Compression and Ignition
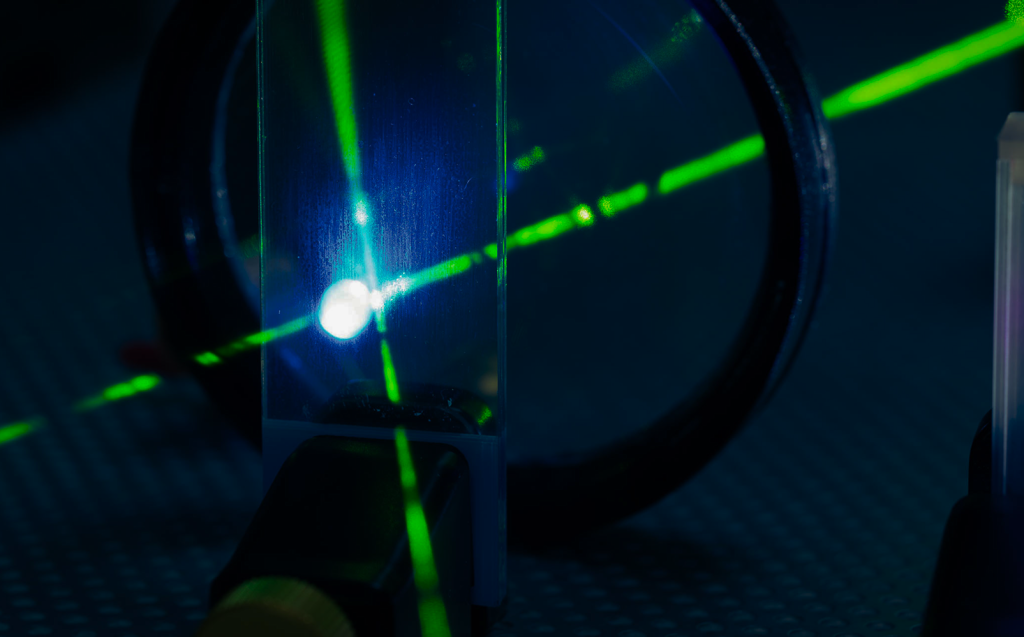
Once the fuel pellet is compressed to extreme pressure and temperature, the equation is set for nuclear fusion. The core of the pellet reaches temperatures exceeding 100 million degrees Celsius, and the pressure becomes millions of times that of Earth’s atmosphere. At these conditions, fusion reactions begin to occur as deuterium and tritium nuclei collide, fuse, and form helium and high-energy neutrons.
The nuclear fusion burn wave then propagates outward from the center of the pellet, spreading the reaction through the remaining compressed fuel. This self-sustaining chain of fusion reactions is what we call ignition. When ignition is achieved, the energy released by the nuclear fusion reactions is enough to continue compressing and heating the remaining fuel without needing additional laser energy.
Ignition is a critical milestone in fusion research because it marks the point where the system produces more energy than it consumes. This self-sustaining process is the key to creating a net-positive energy nuclear fusion reactor, which could revolutionize the world’s energy supply. The nuclear fusion breakthough experiment done at NIF in 2022 reached that goal.
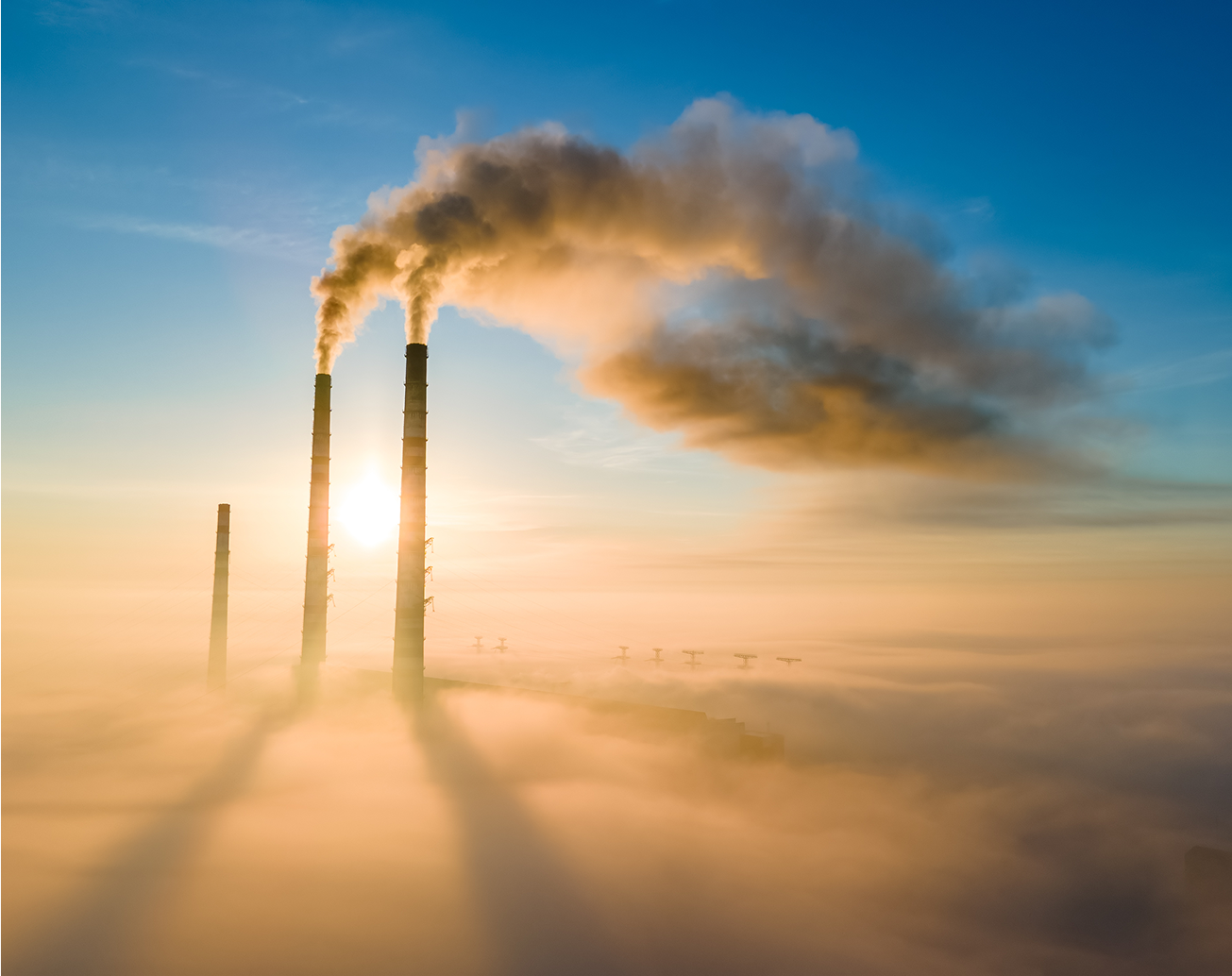
Neutron Capture and Energy Conversion
Once fusion reactions are happening, the next challenge is converting the released energy into usable power. The primary byproduct of deuterium-tritium nuclear fusion is high-energy neutrons, which carry a significant amount of the energy released. These neutrons are absorbed by a blanket surrounding the reactor chamber, typically made of lithium or other materials that can breed tritium (to sustain the pellet fabrication in a closed-loop). The captured neutrons generate heat, which can be transferred to a secondary loop of water to produce steam. This steam can then be used to stoke turbines and generate electricity, much like conventional power plants. The extra heat is cooled down using an external source of cold water. In addition, the blanket material absorbs neutrons and converts their energy, helping to breed more tritium and ensuring a sustainable fuel cycle.
The Advantages of Nuclear fusion over Fission
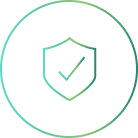
Safety
Unlike fission, nuclear fusion reactions are not self-perpetuating. If the conditions for nuclear fusion are disrupted, the reaction stops immediately. This makes nuclear fusion inherently safer than fission, where a chain reaction can get out of control.
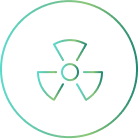
No Long-Lived Radioactive Waste
Nuclear fusion produces minimal radioactive waste. The primary byproduct is helium, a non-toxic, inert gas. The materials used in nuclear fusion reactors may become radioactive, but the waste has much shorter half-lives compared to fission byproducts, which can remain dangerous for thousands of years.
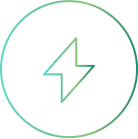
Abundant Fuel Supply
The primary fuels for nuclear fusion, deuterium and tritium, are abundant. Deuterium can be easily extracted from seawater, while tritium can be bred in the reactor itself using lithium. This makes nuclear fusion a highly sustainable energy source with virtually unlimited fuel.
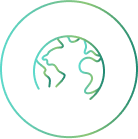
No Carbon Emissions
Nuclear fusion has no impact on air quality a as it doesn’t produce carbon dioxide or other greenhouse gases, making it a clean energy source. This is especially important in the fight against climate change and reducing dependence on fossil fuels.
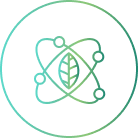
No Risk of Meltdown
In nuclear fusion reactors, there is no possibility of a catastrophic meltdown like those that occurred at Chernobyl or Fukushima. If the reactor were to fail, the nuclear fusion reaction would simply stop. By definition, this makes nuclear fusion safe for environment and biodiversity.
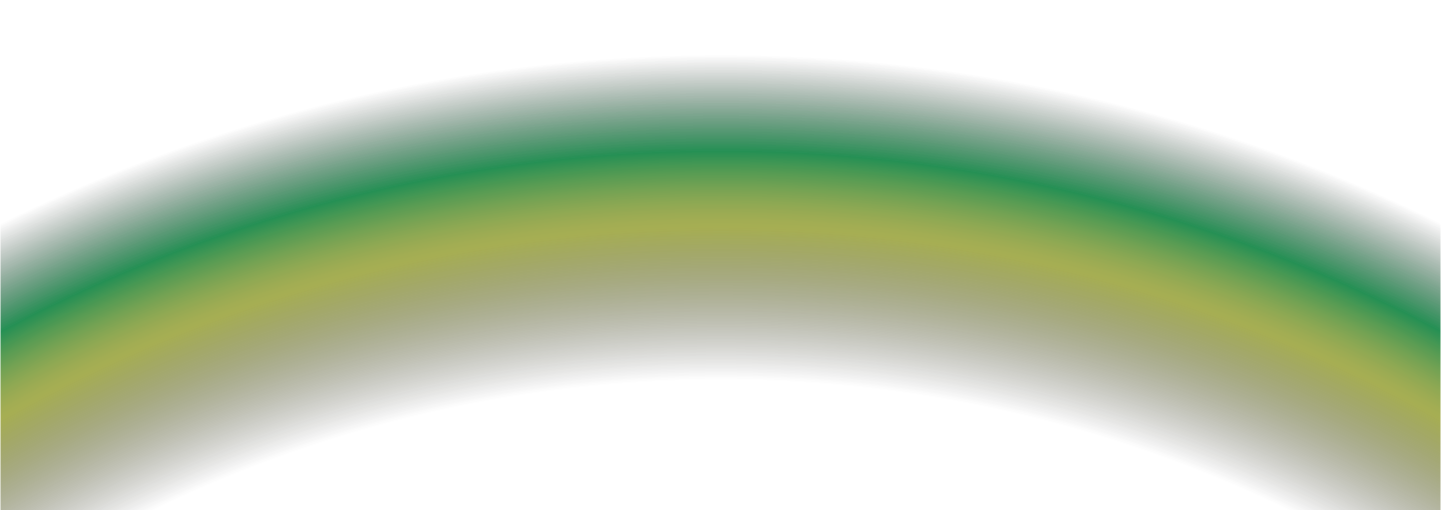
Contact us
Do you have any questions? Don’t hesitate to write to us.
We’re here to help!